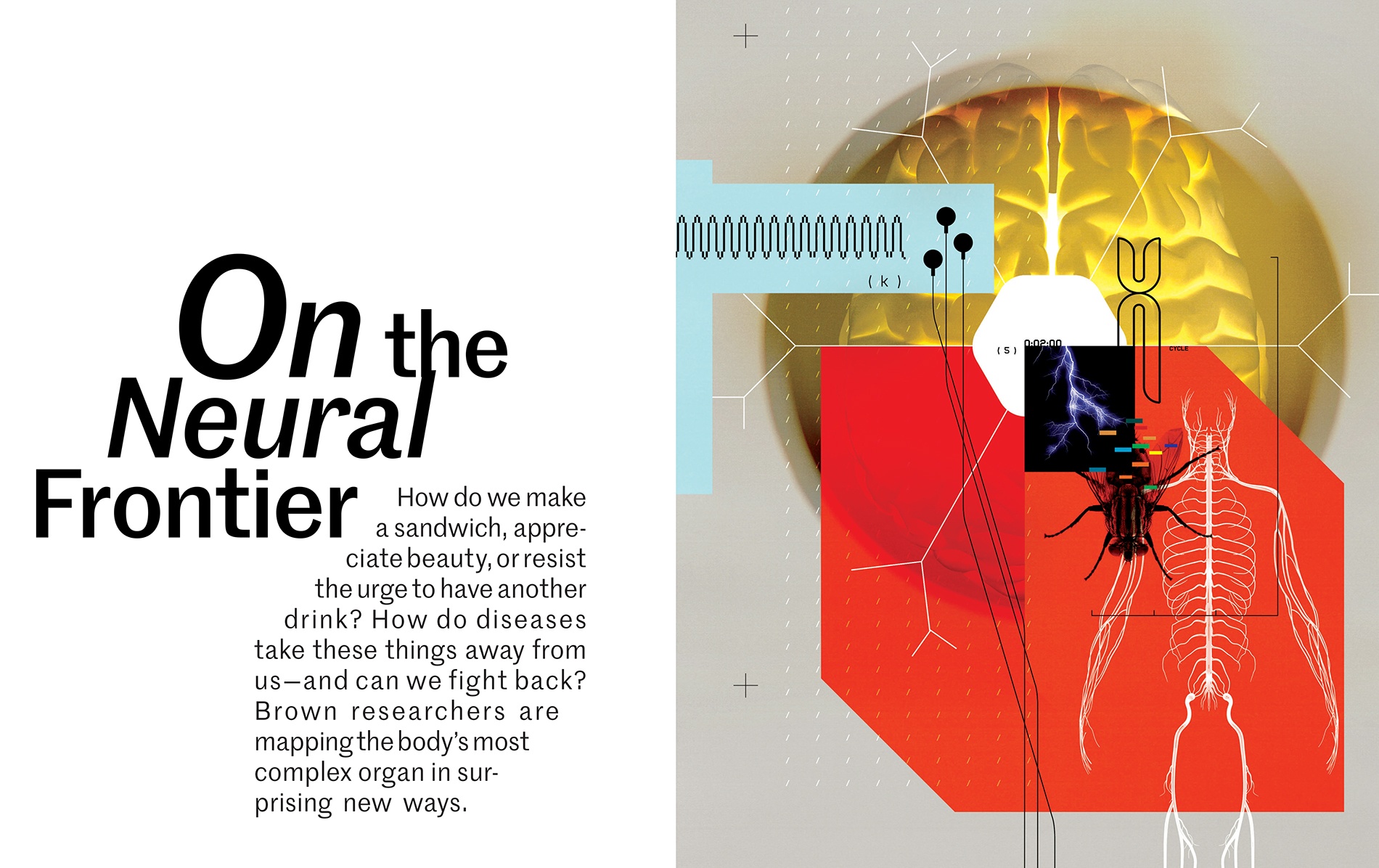
There are worms in the mail. Thousands of them, all wriggling their way alive to the lab of Brown neuroscience professor Anne Hart. “Worms are going by U.S. mail all the time,” Hart says. “FedEx… You have no idea. Worms are tough.” The particular worms Hart works with, C. elegans, are tiny—about the size of the dashes in this sentence—but Hart hopes that their tiny brains might hold the key to unlocking a cure to the neurodegenerative disease Amyotrophic Lateral Sclerosis (ALS). When they arrive, Hart stacks them in her refrigerator in Petri dishes by the hundreds of thousands. Then she takes them out in batches and blasts them with radiation and chemicals to cause random mutations in their genome.
Hart is looking for worms that survive the awful effects of ALS, which gradually causes neurons and muscles to die until the sufferer loses the ability to move. Certain genes, however, seem to prevent those symptoms from emerging even in worms genetically modified to have the disease. Hart is looking for these “suppressor genes” among the 20,000-odd genes that make up C. elegans’s genome, taking survivors and breeding them through multiple generations until she can isolate single alleles that rescue neurons and muscles.
It’s a radically different way of tackling ALS, which researchers usually attack by targeting the gene that causes the disease directly. But Hart’s novel approach has already started to have success. Her lab has so far found more than two dozen genes that seem to suppress the disease—in completely different parts of the genome than anyone has thought to look for them before. And that is just the first step in a multi-year project. Another of her colleagues at Brown is performing the same shotgun blast investigation with fruit flies; others are studying ALS in mice and humans.
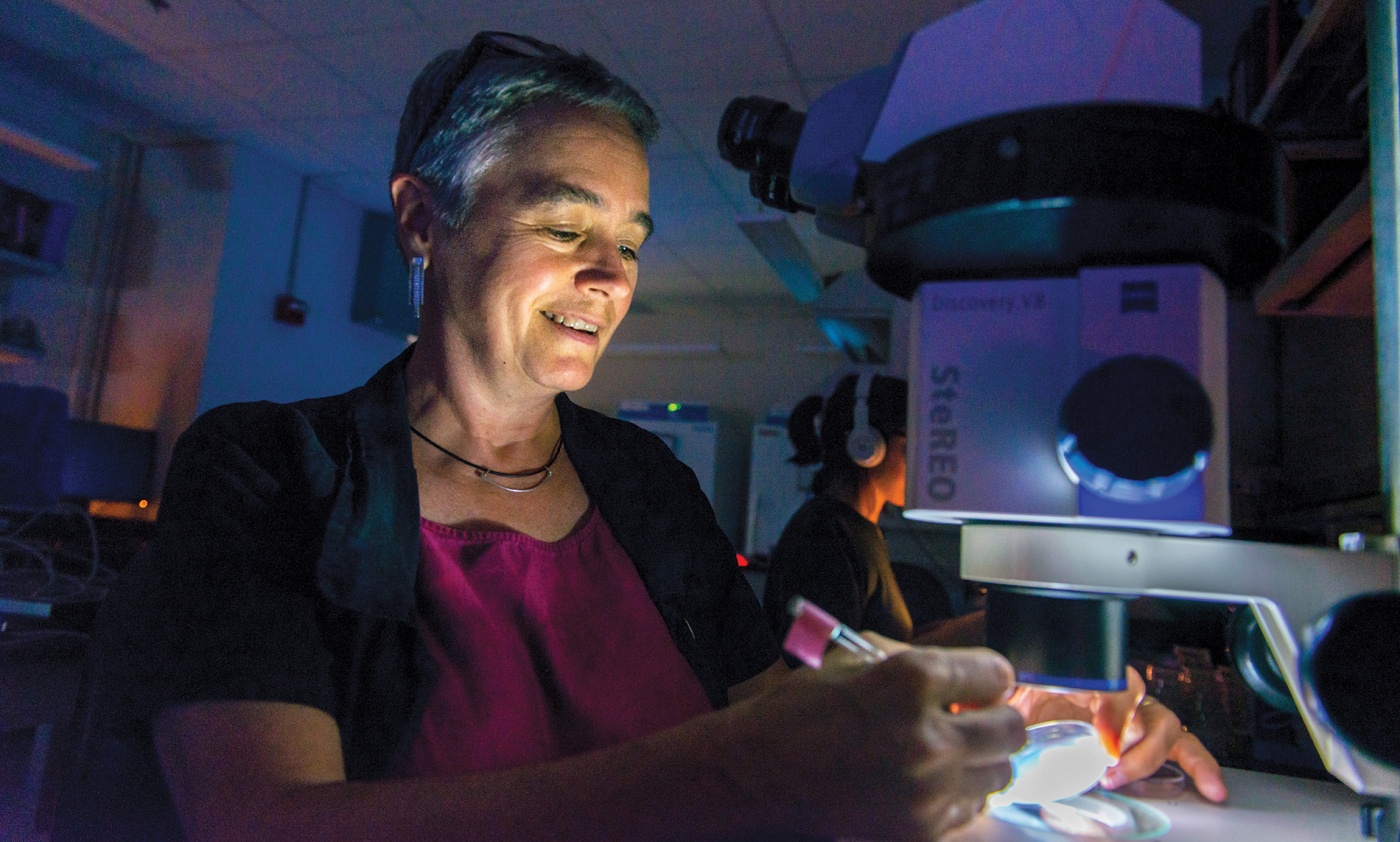
For all of our obvious differences, the genes controlling neuron and muscle survival in all of these species are remarkably similar, says Hart. “If we can find something that stops nerves from dying from ALS in worms and flies and mice, it’s going to work in humans,” Hart says. If it does, the approach could eventually be a model that could be applied to other neurodegenerative diseases, including Parkinson’s, Alzheimer’s, and dementia. “There are no cures or effective therapies for any of them,” Hart says. “If we can show that the worm, fly, mouse strategy works, oddly enough, that may be the most efficient way to find a cure.”
The project is a risky gamble, but it’s one that could reap great rewards. It’s also emblematic of how brain science is practiced at Brown. Even before Houston businessman Robert J. Carney ’61 and his wife Nancy, a former TV news producer, gave $100 million to the Brown Institute for Brain Science this spring, the institute had already become an international leader in brain science, bringing together multiple researchers—and sometimes multiple species—to tackle complex neurological issues. Over the past 20 years, Brown brain researchers have led the way on brain-computer interfaces such as BrainGate to give mobility back to patients with paralysis; pioneered the field of computational neuroscience, which uses mathematics to address behavior and mood disorders; and made breakthroughs in our understanding of neurodegenerative diseases such as ALS and Alzheimer’s. The Carneys’ gift—one of the largest in Brown’s history—instantly boosted public awareness of the renamed Robert J. and Nancy D. Carney Institute for Brain Science as one of the premier brain research institutions in the world, and will allow it to set an ambitious agenda, pursuing cutting-edge techniques for understanding the body’s most complex organ.
“The Carney gift allows us to think big—really big—about taking on these challenges,” says institute director Diane Lipscombe. “It takes massive courage and collective passion from a number of different scientific disciplines to say, ‘I am going to take the leap into this area, and we may not succeed, but we need to provide the funding necessary for people to try.’”
After all, it’s exploratory research that pushes forward the frontiers of knowledge, paying off with breakthroughs that allow us to create technologies and drugs that can cure diseases and heal injuries. With the help of the new gift, the institute will open up its own dedicated space above the Brown Bookstore at 164 Angell Street, building state-of-the-art new labs to bring together researchers in cognitive neuroscience, computational neuroscience, and neuroengineering. In addition, it will give the institute more long-term stability and funds with which to support risky and bold new ideas. “The onus is now on us, and the responsibility is now ours, to do something really big and have an impact,” says Lipscombe. “We won’t do that by being conservative.”
Collaborating to unlock mysteries
Lipscombe, who is also president-elect of the Society for Neuroscience, focuses her own research on ALS, slicing mouse spinal cords into 400-micron sections to identify tiny changes to motor neurons that might provide an early signal to the disease. Combining that research with the gene suppressor work of Hart and other colleagues might one day allow scientists to stop the disease before it starts, by preventing genes from expressing themselves before degenerative symptoms take hold.
That integration of disciplines, says Lipscombe, “is completely essential to really deeply understanding brain function.” Think about it: No other organ in the body has anywhere near the complexity of the brain. The heart is a muscle to pump blood. The lungs are oxygen exchangers. The skin is a big, sweaty sleeve. But the brain somehow fires electrical charges through billions of tiny neurons in order to allow us to move our toes, remember the smell of grandma’s pot roast, and understand Kierkegaard. Even reading a magazine article like this one takes an incredible amount of coordination for the brain, which has to send all of the signals for the eyes to follow the words, the hands to turn (or scroll through) the pages, and the cerebral cortex to make sense of their meaning.
“The brain is what defines us, it’s what makes us laugh and cry and appreciate the beautiful things in life,” says Lipscombe. “Deep down, we all try and understand why we make certain decisions, define who we are, and how we interact on a social level.” And when something goes wrong in the brain, as it does with neurological disorders, we struggle to comprehend the psychological and biological causes of the disease. “Once we can understand and describe and explain it, we will be empowered to bring people [who are ailing] back into the fold and say, ‘This is how we help them.’”
Creating that understanding, of course, means bringing together techniques from many different academic disciplines. “Interdisciplinary” is one of those buzzwords that’s thrown around a lot in academia, almost as overused as “collaboration.” In Carney’s case, however, it’s warranted. Brown created a center for what was once called neural science in the early 1970s. As early as 1999, it created a brain science program as a forum for different disciplines to collaborate, growing into the institute by 2009. Now it stretches between 45 labs across campus, with 130-some professors working in fields as diverse as psychology, neuroengineering, and computational neuroscience.
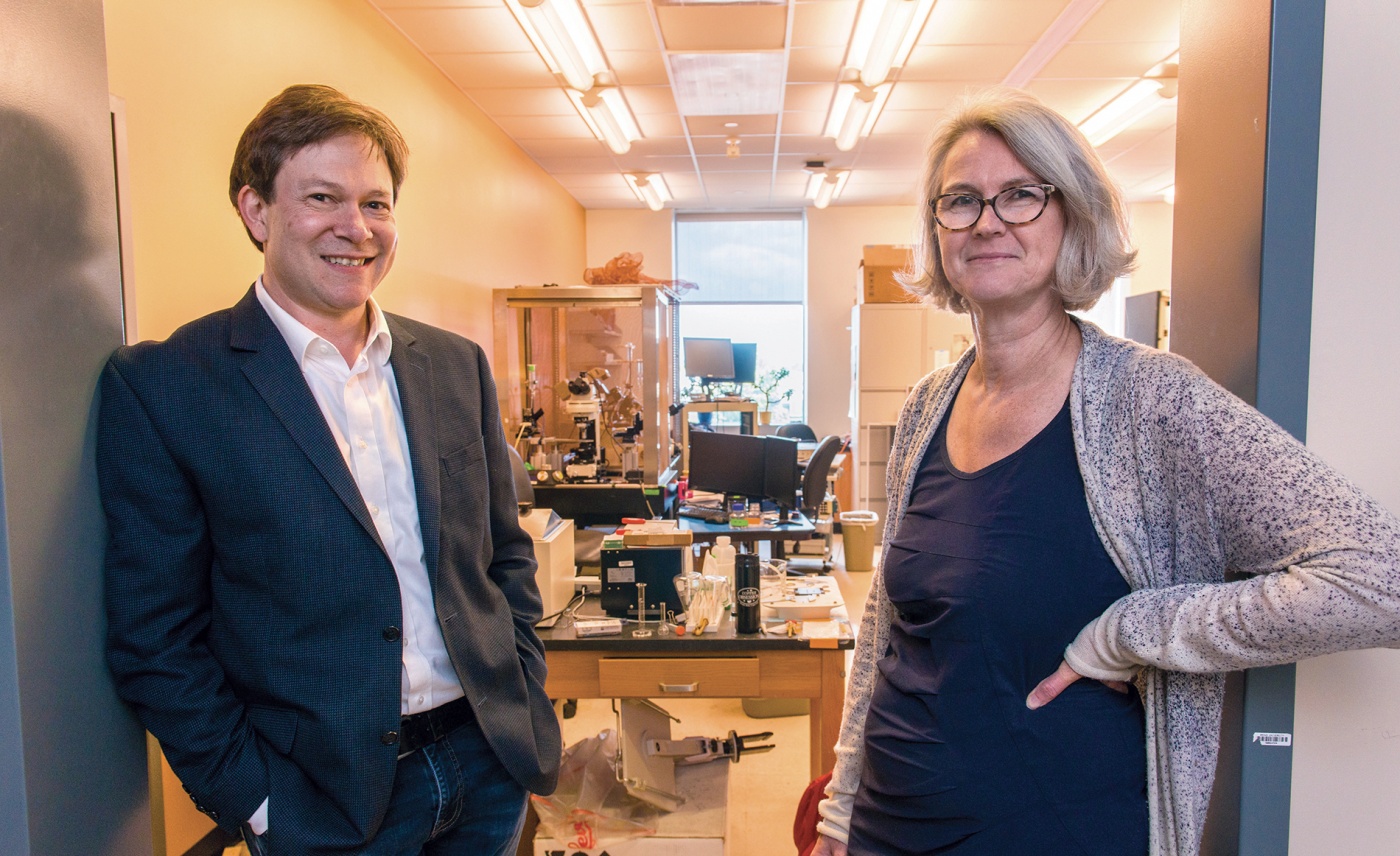
Recently, the institute scientifically codified the extent of its collaborations, according to Chris Moore, a neuroscience professor and associate director of the center, comparing the number of papers published by institute faculty over the past six years to that of two similar brain science institutes at other top schools (which Moore declines to name).
Moore found that there wasn’t a single year in which Brown researchers published the most papers—however, the number of coauthorships with fellow researchers was three times as much as the nearest comp. “That number popped off the page,” says Moore. In addition, every single year, Brown had the most papers cited by other papers in the field. “If we were to make baseball cards, on the back of our card would be ‘more collaborative than most, and better respected.’”
His own research, Moore says, typifies that collaborative approach. Moore studies how the brain processes attention in real time—recognizing, for example, when someone calls your name from across the room, even when you are engaged in a conversation with someone else. To study how the brain accomplishes such feats, Moore’s lab came up with a novel way of tracing signals through the brain using bioluminescence, essentially the same phenomenon that makes fireflies and jellyfish glow. “It’s like putting a lightbulb inside the brain, if you will,” he says.
When he first came up with the idea, however, he didn’t have the ability to test it in his lab. “We said, there is this amazing biophysicist down the hall, but she’s going to think we’re crazy,” he remembers. Knocking on Lipscombe’s door—which was, as it happens, open at the time—he explained the basics of the plan, which had never been tried before. “She listened and said, ‘Have you thought about trying it this way?’” Moore recalls. Together they developed a simple injection that would cause the brain cells to luminesce in the presence of calcium, a marker of brain activity. “Literally within two weeks we had evidence it worked,” he says. “That captures the spirit of collaboration here—instead of saying you are crazy, get out of my office, or I’ll help but here’s the price of collaboration, it was an immediate embrace of an idea.”
The institute helps to spur collaboration by helping researchers apply for external grants as well as administering a half-dozen Innovation Awards—grants of up to $100,000 for early-stage research that is too risky or experimental for government funding. By definition, those kinds of risky endeavors often involve scholars from multiple disciplines. “Forced marriages never work,” says John Davenport, the institute’s managing director, who is in charge of administering grants. “A collaboration is almost always away from the main work someone does—it can be risky to divert energy into something that’s not funded and may not work. We are most successful when we are able to bring together people who want to work together but who find it difficult because of time, distance, or risk.”
Transmitting impulses—wirelessly
The Carney Institute is perhaps best known for its leading role in BrainGate, a 20-plus-year project that creates revolutionary medical devices by implanting electrodes inside the motor cortex of the brain, connected by cables to a computer. Led at Brown by professors Leigh Hochberg ’90 (engineering) and John Donoghue ’79 PhD (neuroscience), the devices capture the brain impulses of patients injured by ALS, stroke, and trauma, and used algorithms to translate them in real time into digital computer signals. Those signals, in turn, have allowed the patients to communicate through a keyboard or to move a mechanical arm just by thinking about it.
The newest goals of the team have been to literally cut the cord, creating less invasive means of achieving the same level of control. “We are trying to get to the point where we can make 24-hour recordings of brain activity, to help people whenever they need it,” says neuroengineer David Borton ’12 PhD, an assistant professor. “That means no cable at all, making it easier for people to go about their daily lives.”
In the current system, the cables pass through the skin and skull as well as through the outer layers of the brain to connect to electrodes sitting directly on the cerebral cortex. In doing so, they break through the meninges, important barriers that protect the soft and sensitive parts of the brain underneath. Although they’ve never been linked to infection, there’s the potential that they could create a highway for bacteria to enter the brain; more prosaically, as with phone chargers, the cables can bend or break with repeated use.
Borton has been working on a new electronic platform that would sit on top of the meninges, with nodes placed beneath—anywhere on the brain—able to record and stimulate hundreds of neurons. These floating electrodes would connect to a computer wirelessly, from a single port behind the ear, which would look similar to a hearing aid. The new system would limit infection and provide greater flexibility in what could be stimulated—and understood. “You can’t understand the brain by looking at just one part,” says Borton. “We need to record from multiple parts of it at the same time, and this platform allows us to do exactly that by getting rid of the cabling problem.”
Neuroscientists have already realized that while there are broad areas of the brain that control, say, attention or visual processing, neurons in other parts of the brain can perform some of these same tasks, making up for other parts of the brain that have been damaged. By being able to record continuously from multiple areas of the brain at once, Borton hopes that, in the future, researchers will be able to better understand individualized disease patterns in brain activity, enabling personal and precise solutions.
Closing in on OCD?
Devices today already take the first step in providing tailored solutions to brain injury and disease. In DBS (deep brain stimulation), researchers implant electrodes that deliver electronic pulses two or three inches inside the brain. Current DBS techniques have been shown to stop the tremor caused by ailments such as Parkinson’s—even if we don’t exactly know why.
“It’s a pretty simple technology—it’s amazing it works as well as it does,” says Brown neurosurgeon Wael Asaad, a DBS specialist who works at Rhode Island Hospital. “A lot of our research is to get smarter about how we use it, and to see if we can modulate stimulation in a more dynamic way in accordance with a patient’s symptoms.”
Asaad does research on animal and human subjects, examining how the brain responds to specific stimuli. To prepare patients for the procedure, a doctor implants two small electrodes deep into the brain, which are connected by wires to a stimulator device typically implanted in the chest. “We do the procedure awake, so we can map out the circuits as they react to stimulation,” he says. “We say, if you are comfortable, could you do motor tasks and have us measure your brain activity? Often, they are not only willing but enthusiastic to help with our research.” (Since there are no pain receptors in the brain, the procedure is not painful for patients once electrodes have been implanted.)
But what if that same device could respond to moment-to-moment fluctuations in neurologic symptoms? With Borton’s help, a large NIH-supported team (including Carney Institute professors Michael Frank and Matt Harrison ’00 ScM, ’05 PhD) is developing a new methodology to treat Obsessive-Compulsive Disorder (OCD). Symptoms of OCD are dependent on environmental cues, like a compulsion to perform a ritual when touching a certain doorknob. The team has an FDA Humanitarian Device Exemption for their treatment, which uses specially developed algorithms to determine when the device should deliver stimulation to reduce compulsive behaviors over time. In particular, the team believes machine learning can be applied to neural signals to improve how stimulation is delivered.
“It’s a good example of how the institute can bring people together,” says Borton. “I knew little about deep brain circuits before we started.” Nevertheless, by applying engineering principles to neuroscience challenges, Borton hopes to demonstrate that device-based therapies can be more precise, and have fewer side effects, than drug-based therapies. Interestingly, the same areas of the brain have also been implicated in depression and anxiety disorder, and so homing in on exactly what area to stimulate to relieve OCD symptoms could help relieve those disorders as well.
Trying to decode patterns
Theresa Desrochers takes a different angle in her approach to OCD. Desrochers, hired in 2016 directly by the Institute (as opposed to one of Brown’s science departments), is a professor of neuroscience and of psychiatry and human behavior. In her lab at Brown, she studies how the brain performs sequences of seemingly simple tasks—for example, making a sandwich.
“If you think about what it takes to make a sandwich, it’s not only remembering the concrete motor patterns,” says Desrochers. Even such a simple task can vary in ways both large and small. “The mustard might be in a different place, or the cold cuts might be in a different place. You have this overarching goal, but you don’t have concrete motor patterns in order to accomplish it.” Even a sophisticated computer might be stymied by the complex choices involved in making a ham-on-rye. Yet, most of us do it so effortlessly, it’s packaged up and in the lunch bag before we even realize we’ve done it.
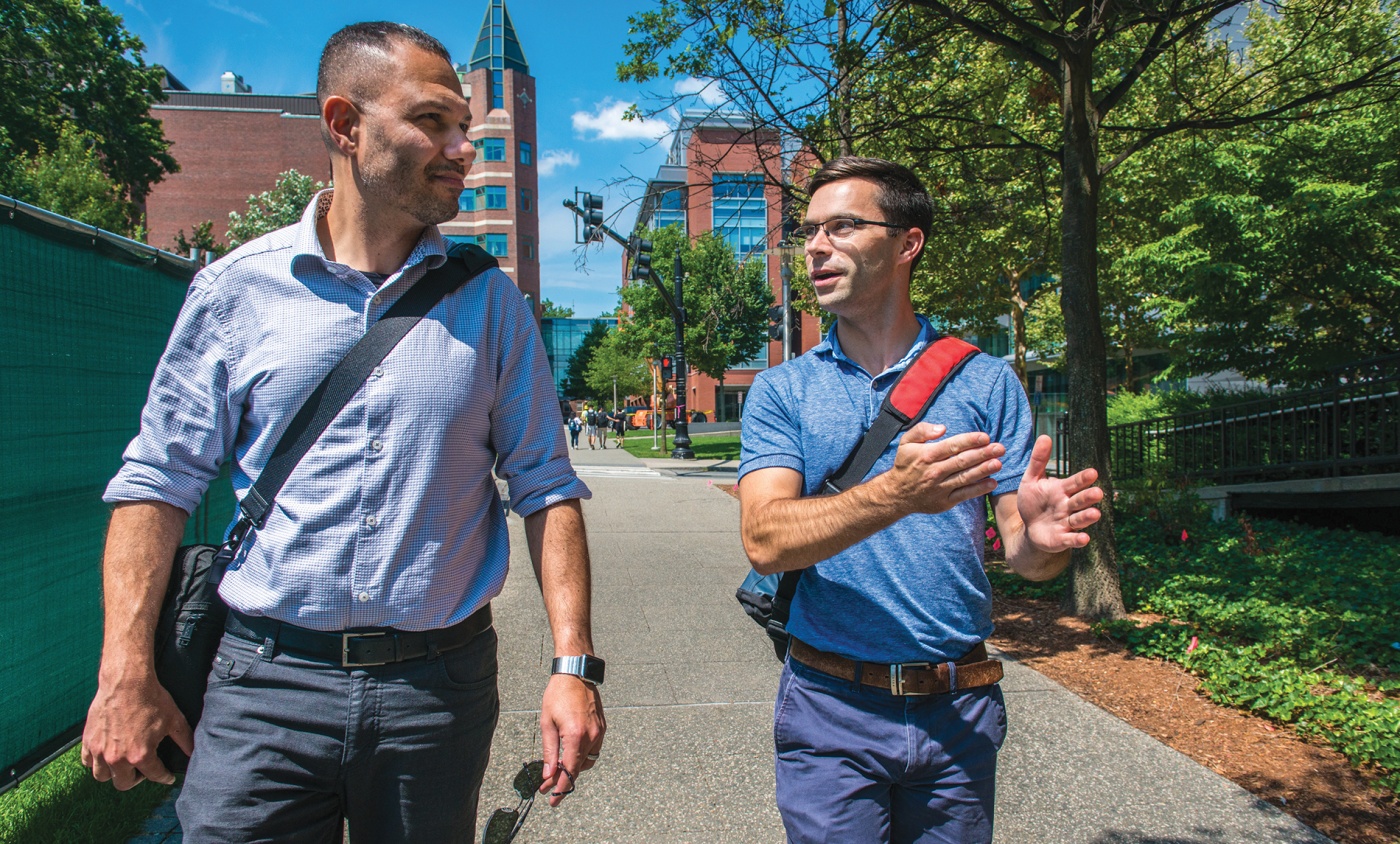
Desrochers has focused her research on breaking down those rote sequences of actions to figure out how the brain actually processes and keeps track of its actions. She’s found that most people break up tasks into specific sequences of actions without even realizing it. In one study, she asks participants to play a game on a computer screen, in which they are shown a series of colored shapes, each one of which can be either a square or a circle, and either red or blue. The program then asks the participant to type a certain key depending on its shape or color.
Desrochers starts with sequences of four shape-color combinations, and asks participants to identify a sequence of four items—for example the first two in terms of color, the second two in terms of shape—repeating the four-item sequence. She has found that for each four-item sequence, brain activity ramps up. That implies that the brain is keeping track internally even in the absence of any external pattern—the computer does not pause between sequences. “You have this internal boundary, even when there’s nothing on the screen telling you that you are restarting,” Desrochers notes. This experiment also measures the brain’s ability to keep track of abstract tasks. There are multiple shapes and colors, so the computer keys the participants have to press vary—there’s no pattern for their fingers to follow. They have to remember the abstract sequence, just as one remembers to put the mustard on the rye, whether the mustard is located in the fridge or in the cabinet.
Desrochers has focused her experiments on a part of the brain called the rostrolateral prefrontal cortex, which she believes keeps track of this sequencing. In some experiments, she’s disrupted that area with transcranial magnetic stimulation, a way of noninvasively stimulating the brain with a magnetic pulse, and found it causes people to make mistakes. “This might give us a better understanding of where we should look in the brain for circuitry that has gone awry,” Desrochers says—both in people with diseases and in those of us who are getting older and starting to have trouble keeping track of things.
Desrochers hypothesizes that issues in this area might be involved in OCD, causing people difficulties in starting or ending sequences and leading to compulsive behavior. She is now working with psychiatry chair Steven Rasmussen ’74, ’77 MMS, ’77 MD, who is running a huge battery of tests on patients with OCD and has agreed to include one of her experiments. By comparing results for participants with and without OCD, she might gain insight into how this region of the brain could influence the disorder.
“Having these ideas of what hypothetically might be going on in terms of circuitry, and then actually being able to test these hypotheses, is really amazing,” she says.
Reading brain currents
In the past five years, there has been an explosion of new tools that researchers have developed in order to better understand the geography of brain signaling. Neuroscience professor Stephanie Jones was originally trained in mathematics but switched to neuroscience halfway through her PhD after hearing a talk about how math could be used to trace electrical signals in the brain. “I just realized, ‘Wow, I can really use the math I’ve learned to make a difference.”
She focused on the burgeoning field of computational neuroscience, which uses complex mathematical calculations to understand the brain. Since Jones joined Brown seven years ago, the Carney Institute has become a leader in the field, which bridges math and science.
Every nerve cell has an input and output pathway: one consists of the spindly dendrites that bring electrical charges into the cell; the other is the long cord of the axon that brings charges out of it. By measuring the amount of charge along this pathway, Jones is able to create a model of coupled differential equations that reproduces cell communication. Using many such equations, she is able to follow the charges through different cells to trace the path of a circuit, like electricity whizzing through a cable.
In order to take those measurements, Jones has focused on certain cells with extraordinarily long dendrites that go all the way across the layers of the cortex, the outside shell of the brain. “These long dendrites are aligned in such a way that they are just like telephone poles,” Jones says, “and so they create this big electrical current, big enough to record outside the head.”
Using that concept, Jones’s lab has developed an open-source software called Human Neocortical Neurosolver. It allows researchers to take the macro-level readouts that they get from EEG (electroencephalography) brain scans and uncover the individual paths generating electric signals during healthy behaviors and during neuropathologies. Part of Jones’s research follows particular brain signals called beta waves, which are involved in forming perceptions and actions. In Parkinson’s disease, beta waves overload brain circuitry, corresponding with difficulties in movement. Jones is now collaborating with Asaad to better trace those circuits, in order to pinpoint where stimulation can be applied to alleviate motor disorders such as tremors.
The secrets of drunken flies
Techniques like fMRI (functional magnetic resonance imaging, which looks at blood flow in the brain) and EEG can give scientists valuable insights into large-scale brain patterns. Electrophysiology and molecular biology give insight into individual neurons. What’s missing: a way to study how individual cells are talking to one another. Neuroscientist Gilad Barnea compares the phenomenon to a phone conversation between two people. “You know someone talked about something, but you only know a part of the story,” he says. “If you know who talked with whom, that gives more context.”
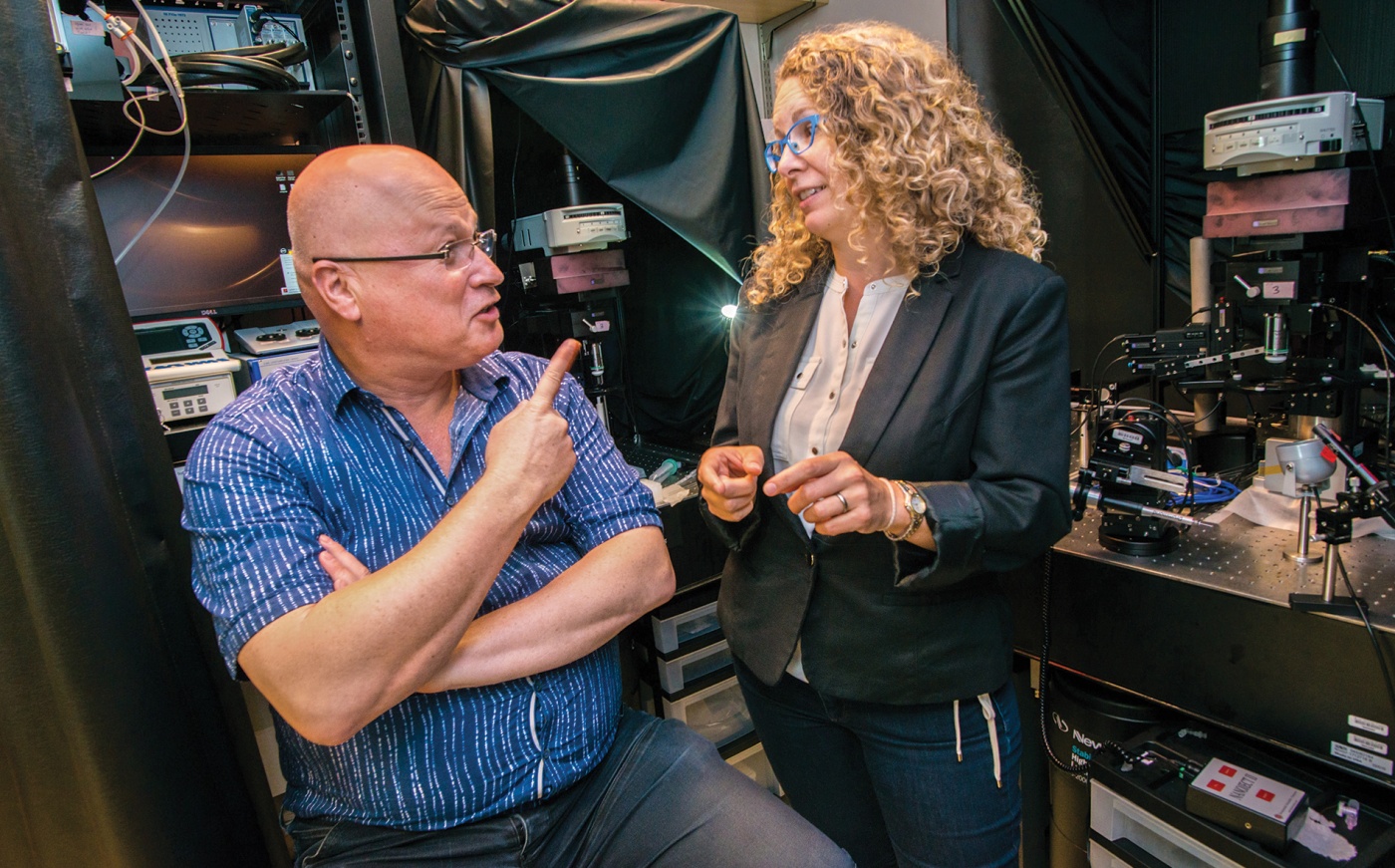
For the past 20 years, Barnea and his team have been developing a new technique, trans-Tango, which helps map brain circuitry in animals, including mice and flies, in ways that might translate to humans. The technique addresses the elusive intermediate level between the activity of individual cells and the brain as a whole, allowing researchers to follow electrical impulses from neuron to neuron.
Barnea uses genetic engineering to implant a “switch” in all of a fly’s neurons that causes them to fluoresce when exposed to a certain protein. Expression of this trigger protein in a “starter” neuron then reveals all of the neurons that are connected to it, lighting up a path through the brain.
Barnea has been using the technique to examine how the brain processes taste and smell, but theoretically it could be used to trace any circuit. For example, the team is currently using trans-Tango to study the circuits underlying sexual behavior and light avoidance, to better understand what flies are “thinking” when exposed to different situations. These studies could ultimately help explore neural pathways in the human brain—and help doctors intervene when they go awry in various neurological and psychiatric disorders.
Trans-Tango is just one of many techniques that Karla Kaun, the Robert J. and Nancy D. Carney Assistant Professor of Neuroscience, is using to map which brain circuits are involved in various behaviors and how experience may change them. In particular, her lab examines the circuitry of alcohol addiction and molecular mechanisms underlying memory and addiction. She hopes her research can illuminate where addictive cravings come from, and why they are so hard to beat.
In one experiment, Kaun exposes flies to an alcohol vapor that affects their brains much like a couple of martinis affect humans. “They will start bumping into each other and courting indiscriminately,” she says. “After a little more, they’ll start to stumble, and eventually they’ll pass out.”
While this process is happening, Kaun takes individual flies and places a tiny two-photon microscope onto their heads. Shining a laser onto their brains, she can trace the flow of calcium, an indicator of brain activity. The research shows that exposure to alcohol actually changes which genes become modified in reward circuits—changing the strength of memories as they are being made.
This mechanism, she believes, may strengthen a future desire for the drug. For instance, people who abuse alcohol often report that there’s a particular bar or restaurant, or even a type of glass, that creates a powerful desire to drink years after they’ve quit. “You can treat physical symptoms, but the hardest thing to overcome with addiction is the craving,” Kaun says.
Recently, Kaun has been collaborating with Barnea to use trans-Tango on flies to trace the circuitry of flies’ memory circuits even more precisely. “We can map all of the neurons associated with memory formation from start to finish, from experiencing that sensory stimulus to making these behavioral choices,” she says. “That has never been done before.” By better understanding those mechanisms, her research may one day lead to drugs in humans that could short-circuit these pathways, weakening those memories and curtailing the desire to drink.
Kaun is grateful for the freedom working at the Carney Institute offers. “It provides me the opportunity to work on these basic neuroscience questions without having to worry too much about translational impact,” she says. In other words, she can delve into understanding how the brain works without being pressured to come up with a profit-making drug or medical procedure. “As an assistant professor it’s rare to have a title, and as a woman it’s even rarer,” she observes. “It means so much to me that the institute values my work.”
Coffee and breakthroughs
For the Carney Institute, the recent $100 million gift made a difference before a penny was spent. Brown may be a leader in brain science, but people tended to be aware of researchers as individuals. “The Carneys’ gift drew people’s attention,” says Lipscombe. At an international brain science conference in Berline, “all the European people were talking about it.” She kept on hearing people say, “I didn’t realize [insert name of prominent brain scientist] was at Brown.”
Lipscombe is particularly excited about how the infusion of resources will help realize the institute’s vision of an even more collaborative and interdisciplinary approach to brain research. The newly renovated space above the Brown Bookstore—plans are to move in next year—will literally sit researchers from engineering, neuroscience, and cognitive, linguistic, and psychological sciences right next to one another. “It’s a bit of an experiment,” Lipscombe says with a smile, though the faculty involved already work collaboratively and are excited about the shared-space model. “Proximity matters,” says Lipscombe. “If you’re sitting down and having a cup of coffee and you’re just chatting about your research, projects emerge from that; collaborative ideas emerge from that. And they are the kind of ideas that you couldn’t ever conceive individually.”
Lipscombe is also excited about her newfound ability to aggressively recruit young talent and about increased collaboration with the Warren Alpert Medical School. The Carney Institute and the Brown Institute for Translational Science—the folks who take research discoveries and try to translate them into treatment options—are currently in discussions about a collaboration in the area of Alzheimer’s and neurodegeneration.
“It’s a little boring if you surround yourself with people who know things you know,” says Lipscombe. “Where the exciting things happen is when you surround yourself with people who know things you don’t.”
Michael Blanding is an award-winning investigative reporter and bestselling author of The Map Thief.